Published On September 22, 2013
XIAOWEI ZHUANG COULDN’T BELIEVE WHAT SHE WAS SEEING. Utilizing super-resolution microscopy techniques she had developed, Zhuang, a Harvard University biophysicist and chemical biologist, was trying to fill in missing details of how neurons connect—particularly ambitious, considering the high density and small size of neural structures, which may measure in mere tens of nanometers (one nanometer equals one billionth of a meter).
Zhuang wanted to use STORM (stochastic optical reconstruction microscopy) to zoom in on the architecture of actin at the synapses, where information is passed from one neuron to the next. Actin is an important protein in a cell’s cytoskeleton, which supports its shape. So her lab tagged actin proteins with fluorescent dyes to make them glow against the black background of the nerve cells. But Zhuang and her team were distracted by a weird pattern in the actin inside the axon, the long arm of the neuron. Actin usually occurs as long or branching filaments, but here it formed rings spaced at regular intervals by a rod-shaped protein called spectrin. A unique latticework column defined the inner circumference of the axon.
Zhuang and her team showed that the column may help organize sodium channels that produce the spiking electrical signals that cause neurons to “fire” signals to other neurons. They also speculated that it may give the axon needed strength and flexibility to maintain its integrity as the body moves around. In January 2013, Zhuang published this work in Science, noting another lab’s discovery that deleting the gene for spectrin in worms leads to broken axons.
“It was an epiphany,” says Vann Bennett, a professor in the departments of cell biology, biochemistry and neurobiology at Duke University, who discovered spectrin in neurons. He wonders whether this might explain why motor neurons, which have very long axons, suffer most in neurodegenerative diseases such as amyotrophic lateral sclerosis. Perhaps this newly discovered skeletal support in the axon breaks down in those disorders. “It’s a question worth investigating,” he says.
That’s a major benefit of super-resolution microscopy—it sends researchers in pursuit of answers to questions no one had previously known enough to ask. That has been the magic of the microscope, ever since Antonie van Leeuwenhoek produced the first magnifying device capable of revealing single cells in 1674. Then, people were astounded to see previously invisible “animalcules” (bacteria) swimming in a drop of water. Now we can observe proteins scurrying around a cell’s cytoplasm, lipids diffusing through the cell membrane, DNA spiraling in the nucleus, and much more.
In the process, researchers are finding that many of their cell models are much too simplistic. “We have cartoon concepts based on assumptions we couldn’t test because they explained things that couldn’t be observed,” says Jennifer Lippincott-Schwartz, a cell biologist at the National Institute of Child Health and Human Development in Bethesda, who helped develop a super-resolution imaging technique, called PALM, for photo-activated localization microscopy. “Super-resolution microscopy can help bring these concepts to maturity.”
UNTIL RECENTLY, microscopes worked by detecting light that reflected off an object. But about 15 years ago, fluorescent proteins and dyes revolutionized the field. Fluorescent molecules absorb light (photons) from a light source such as a laser, and then emit the photons so that the radiating molecules themselves are the source of visible light. Researchers can tag parts of a cell with a rainbow of fluorescent molecules to produce gorgeous still and moving images of brightly glowing, color-coded molecules.
Yet even fluorescent microscopy, by itself, couldn’t reveal biology’s deepest secrets. In microscopy, resolution refers to the shortest distance between two points at which each can be distinguished. But there has always seemed to be a physical restriction on how narrow that space can be. Because of what’s known as the diffraction limit of light, objects closer than about 200 nanometers (about half the wavelength of the standard light used to take images) couldn’t be individually identified. In a cell’s bustling interior, components of important structures—the plasma membrane, energy-producing mitochondria, signal-receiving and -transmitting receptors, and many disease-causing microorganisms—are all much too dense to see individually because of this diffraction barrier.
Super-resolution optical microscopy can resolve these and many other structures, and if they’re fluorescently labeled, it can identify exactly what each molecule is doing. Developed in 2005 and 2006, various techniques use “tricks” to resolve objects from two to 20 times as small as can be seen with conventional fluorescent microscopy.
STORM and PALM use “photoactivatable” fluorescent molecules that can emit a lot of light but that can also change—blinking from dim to bright or from one color to another color—when exposed to particular wavelengths. Instead of shining a bright laser that activates all fluorescent molecules at once, as with standard microscopy, super-resolution techniques employ a low-power laser that provides just enough light to activate a random subset of fluorescent molecules. With sufficient iterations, enough molecules will glow for their combined image to form a complete, detailed structure.
Imagine that fluorescently tagged actin proteins represent car headlights and you take a photo of traffic at night from overhead. Conventional fluorescent microscopy turns on every car’s headlights (that is, all the actin proteins glow at once), and you essentially see a time-lapse photo of the traffic patterns. That gives you a very good idea of the overall structure of the area—you can learn a lot from seeing the shape of the actin structures within the cell. Yet this view can’t detect nuances about how a specific actin protein interacts with another protein, or the differences in behavior among actin proteins.
With STORM and PALM, however, headlights blink on and off randomly, and they’re far enough apart to be distinguished as separate glows. Applying an algorithm to the picture, you can locate the center of each glow and see exactly where a single protein is. After one set of fluorescent molecules blinks off, another blinks on, and a succession of pictures of different sets lets you compute the location of all of the proteins. Finally, combining the computed locations of each glow from all of the pictures transforms the streaks of light into discrete dots showing each individually identifiable protein in sharp focus even though the distance between the proteins is smaller than the diffraction limit.
SO FAR, SUPER-RESOLUTION MICROSCOPY has confirmed some assumptions and overthrown others, and in a few cases has revealed something unanticipated. In one study, for example, Lippincott-Schwartz combined PALM with a super-resolution imaging technology called SIM (for structured illumination microscopy), which merely doubles the resolution of conventional fluorescent microscopy. But for many purposes, it yields sufficient detail, and at a wider perspective that provides more context. Lippincott-Schwartz wanted to determine how cells crawl along a surface. So she first used SIM to observe the cell membrane flattening and bulging out into a protrusion that appeared to pull the rest of the cell behind it. Then she looked more closely with PALM to learn how those protruding feet, or lamella, form. Their creeping action is crucial during development, when cells migrate to form differentiated tissues and organs. This “cell motility” also brings cells to repair wounds, and it’s how cancer cells metastasize to healthy tissue.
Scientists had assumed that cell motility depended on a protein machine that formed at the bottom of the lamella and pushed outward. But Lippincott-Schwartz, using SIM, watched arcs of actin filaments forming at the top of the cell and connecting to adhesion points on the surface. These connections are flexed by myosin II, a well-studied protein in muscle cells. By drilling down with PALM, she discovered that myosin II works the same way to drive the contractions in a crawling cell as it does to flex muscle fibers. Pulling back again for live imaging of the crawling appendage using SIM, she watched myosin II squeezing the top surface of the cells as it moved up along fibers that rim the top of the cell-like cables. The cables get squeezed by the motor, which flattens the leading edge of the cell into a shape that enables it to crawl. In other words, the mechanism for cell motility is the same as the one utilized for muscle motion—a connection that could mean that studies in one area might also be relevant in the other.
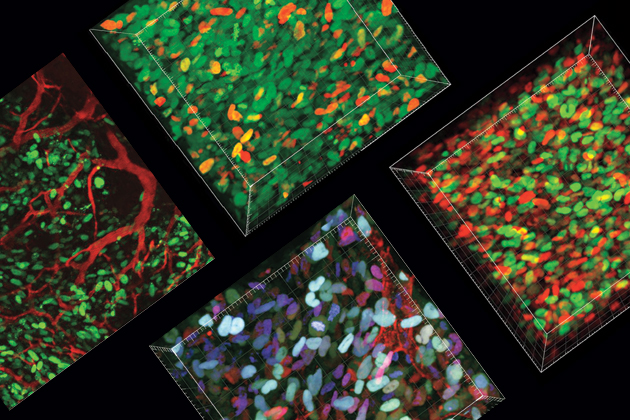
COURTESY OF CENTER FOR SYSTEMS BIOLOGY, MGH WEISSLEDER LAB
AT HARVARD UNIVERSITY, assistant professor of molecular and cellular biology Ethan Garner hopes that his innovative use of single molecule studies similar to PALM and STORM may eventually have therapeutic applications. He uses his approach, called single molecule tracking, to study structures inside bacteria—in particular, to see how the rod-shaped Bacillusbacterium builds and maintains its cell wall. Many antibiotics kill bacteria by stopping the wall from growing, and when bacteria become resistant to antibiotics, they recover the ability to grow their walls. In light of today’s problems with multi-drug-resistant superbugs, Garner says, “my hope is that if we can understand the details of the system piece by piece, we can figure out where the weak points are.”
Garner wants to observe one protein at a time and follow its interactions with other proteins. That requires turning on a single fluorescent protein and monitoring it to create a time-lapse photo. He hopes to track the behavior of enzymes that rotate around the cell and pull short, actin-like cytoskeletal filaments behind them as they synthesize the cell wall.
As a postdoctoral student in 2011, Garner genetically modified those enzymes, or motors, to create a photoactivatable fluorescent protein that normally glows green, but that will turn red when hit by a purple laser. When he turned on a purple laser at a very low level, a single red molecule slowly appeared and he followed it for as long as 30 to 40 seconds. Then he tracked another enzyme. In separate experiments, he followed the filaments that are also part of the wall-synthesizing machinery.
Some enzymes slowly moved across the cell wall in straight lines, rotating around the curved part of the rod, with filaments in tow—a sign that they were synthesizing the wall. Other enzymes diffused with no filaments in their wake, signaling inactivity. When antibiotics were dropped on bacteria, the active enzymes instantly froze up, the cell walls gradually lost their rigidity, and the cells became amorphous blobs. The same thing happened when Garner fed the Bacillus compounds that can turn on or off genes that regulate the enzymes. When the genes returned to their normal status, the enzymes started spinning again and the bacterium snapped back into a rod shape.
“We confirmed what people had thought for a long time, but we may be the first to actually see it,” Garner says. “We are also seeing things that disprove previous theories.” For example, the actin filaments associated with the cell-wall machinery had been thought to be one large structure. Garner instead has seen small, individual filaments with their motors right next to each other, moving in parallel lines, but going both backward and forward. “The reality is much more complex and intricate than our models predicted,” he says. “We always assume it will be the simplest solution, but biology doesn’t care about simplicity.”
As exciting as super-resolution fluorescent microscopy has been, most studies have used cultured human cells that are genetically identical to one another, says Ralph Weissleder, director of the Center for Systems Biology at Massachusetts General Hospital. “Those cells are only model systems and often bear little resemblance to cells taken from a patient, or even from a mouse.” Weissleder, whose research is geared toward improving imaging of cells and tissues in patients, thinks super-resolution imaging is still a long way from being used for such purposes in the clinic.
And there may be issues even in basic research. One is that imaging techniques themselves may alter the physiology of the cell. Eric Betzig, a physicist at the HHMI Janelia Farm Research Campus in Virginia, says that might happen, for example, because of the intense light used in some super-resolution techniques. Moreover, PALM and STORM rely heavily on algorithms and statistics; when you see something novel or strange, there’s a possibility that it could result from your statistical method rather than an actual characteristic of the cell.
“Just because you see it doesn’t mean it’s real,” says Timothy Mitchison, a systems biologist at Harvard Medical School. That’s why it’s important to try to confirm any findings from super-resolution fluorescent microscopy through other methods. More reliable images may result from super-resolution techniques that use fewer statistical tricks and more optical physics, suggests Mitchison. SIM, which Lippincott-Schwartz used in the cell motility study, is one of those, and another is STED (stimulated emission depletion), which uses sophisticated optics and quantum physics to restrict emission from the light scanning the object. That sharpens the focal spot to a dot of light smaller than the diffraction limit, down to about 10 nanometers. But STED is difficult for biologists to operate, has only one color, and is rarely used on living cells.
Despite its shortcomings, super-resolution microscopy does reveal the architecture of cells and molecules in intricate detail. A focus of Lippincott-Schwartz’s research is to observe how viral proteins assemble themselves on cell surfaces, budding off as an intact virus, and also how proteins reconfigure themselves to drive viral budding—knowledge that is fundamental for understanding how viruses cause infection and how to prevent it.
To learn such things, researchers need better, brighter, faster-blinking fluorescent molecules. They need hardware and software that’s easier to use and less expensive. They need optics that delve deeper into tissues and organs without disrupting normal biological activity. And researchers must undertake corroborating studies before leaping to conclusions based on stunning first impressions.
Bennett, the Duke neuroscientist, has compared super-resolution microscopy to “the telescope of Galileo.” Just as that invention revolutionized our view of Earth’s place in the universe, this new optical tool may revolutionize what we know about our molecular selves.
Dossier
“Breaking the Diffraction Barrier: Super-Resolution Imaging of Cells,” by Bo Huang, Hazen Babcock and Xiaowei Zhuang, Cell, Dec. 23, 2010. This review describes the challenge of beating the diffraction limit of resolution and the quantum leap forward in fluorescent microscopy in the 2000s with the development of super-resolution microscopy.
“Putting Super-Resolution Fluorescence Microscopy to Work,” by Jennifer Lippincott-Schwartz and Suliana Manley, Nature Methods, January 2009. The authors discuss how super-resolution microscopy can revolutionize the understanding of the cell, but caution that researchers must still consider the technology’s limitations.
“Actin, Spectrin, and Associated Proteins Form a Periodic Cytoskeletal Structure in Axons,” by Ke Xu, Guisheng Zhong and Xiaowei Zhuang,Science, Jan. 25, 2013. An account of the Zhuang lab’s discovery of a surprising structure in the cytoskeleton on neural axons using the super-resolution technique STORM—opening up questions about neurobiology no one knew to ask before.
Stay on the frontiers of medicine
Related Stories
- Bubble Power
Aiming tiny bursting bubbles at tumors could pave the way for new treatments.
- In One Breath
The air you exhale carries a wealth of clinical data, and scientists are fashioning ever more precise methods for divining its truths.
- Organs in Miniature
Microscopic models—half living, half not—may prove more reliable than animals in explaining human disease and testing therapies.